The redox landscape of SARS-CoV-2 infection
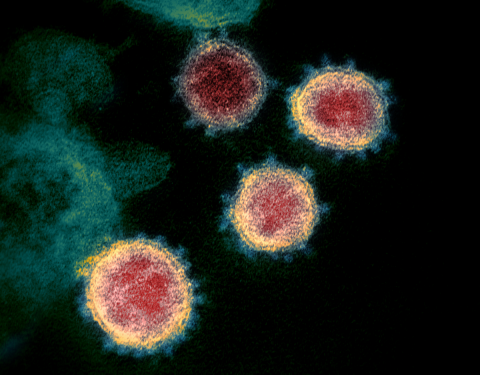
Main Article by Flávia Meotti (Instituto de Química da USP) and Francisco R. M. Laurindo (Incor, Faculdade de Medicina da USP)
The challenge imposed by Covid-19 pandemics has promoted the union of scientists from different fields and technologies, breaking geographic frontiers to solve relevant problems. The focus is, of course, understanding SARS-CoV-2 pathobiology and the ensuing development of improved diagnostic procedures, vaccines and therapeutic compounds. Redox processes certainly belong to this story. In fact, redox pathways can be identified at essentially every level of SARS-CoV-2-related disease and hold a unique potential to integrate knowledge at different systems levels and connect multiple disciplines. Here we briefly highlight, on a somewhat arbitrary and incomplete fashion, redox phenomena of potential significance to this scenario. We hope to inspire our readers to complete this picture and pursue them in depth.
Viral entry into cells: redox and non-redox pathways
The entry of enveloped viruses such as SARS-CoV-2 into cells is a two-step process involving: (1) viral particle binding to cell-surface receptors and (2) fusion of the virion to host cell membranes.
SARS-CoV-2 binding and subsequent entry into the host cell depends essentially on the viral Spike glycoprotein (S glycoprotein). The S glycoprotein forms the characteristic corona of large distinctive spikes in the viral envelope [Xiao et al., 2003]. S glycoprotein contains the S1 domain, a globular region of the protein distal to the virus membrane and the S2 domain, which forms the stalk. S1 domain mediates high-affinity binding with the major (though not exclusive) primary cell receptor, angiotensin-converting enzyme 2, ACE2. Both glycoprotein S and ACE2 exhibit reactive cysteine residues. Membrane fusion is the result of conformational changes in virion envelope depending on its interaction with cells. For SARS-CoV-2, docking to the ACE2 induces conformational changes required for membrane fusion. For enveloped viruses including Newcastle Virus, HIV and many others, thiol/disulfide rearrangements, commented below, are essential for fusogenic activity [Fenouillet et al., 2007; Jain et al., 2008]. For the viral glycoproteins from these viruses, fusion-associated conformational changes are followed and/or preceded by disulfide bond exchanges. This could also be expected for S glycoproteins from both SARS-CoV and SARS-CoV-2, since they contain 39 Cys residues. Following this hypothesis, a series of point mutations replacing cysteines for alanines in S glycoproteins from SARS-CoV revealed that five of seven Cys present at the S1 receptor binding site are essential for association to ACE2 [Wong et al., 2004]. However, the binding of S glycoprotein to ACE2 and infection of mammal cells were largely insensitive to reducing, oxidizing or alkylating agents [Fenouillet et al., 2007; Lavillette et al., 2006]. Rather, for influenza viruses, fusion events have been linked to other stressful events such as cell acidification. These data led to the conclusion that both binding to ACE2 and the fusion capacity of the spike complex are independent of redox switches, in singular contrast to the situation described for a number of other enveloped viruses. However, recent computational simulations indicate that the affinity of SARS-CoV-2 spike protein for ACE2 is strongly impaired when the cysteines present in both proteins (not in only one of them) are in the reduced state, probably due to conformational changes, mainly in ACE2, that affect binding interaction. In contrast, binding is less affected under more oxidizing conditions able to preserve disulfides [Hati & Bhattacharyya, 2020].
Despite being poorly modulated by redox switches, cysteines present at the C-terminal region of the S2 domain undergo post-translational modifications that are crucial to virus infection. Nine of the thirty-nine cysteines in S glycoprotein are clustered between the membrane spanning domain and the C-terminal cytoplasmic tail, with six of these residues being well conserved among different coronaviruses. In SARS-CoV S glycoprotein, the cysteines clustered near to the predicted transmembrane domain were palmitoylated. Mutations of different groups of cysteines significantly decreased protein palmitoylation and virus fusion into mammal cells [Petit et al., 2007]. Since SARS-CoV-2 S glycoprotein shares 79.6% sequence identity with S glycoprotein from SARS-CoV and both contain cysteine clusters at the C-terminal tail, palmitoylation might be important for SARS-CoV-2 fusion as well.
Other viral proteins important for viral replication can exhibit redox modulation. The Nsp9 (non-structural protein 9) from SARS-CoV forms disulfide-linked homodimers important for RNA binding. These dimers assemble in complex quaternary structure. However, cysteine mutations do not impede oligomerization, indicating redundant redox and non-redox mechanisms for RNA binding [Ponnusamy et al., 2008].
Conformational rearrangements of viral envelope glycoproteins by redox reshuffling during virus-cell interaction and fusion have been described in some coronaviruses and many other enveloped viruses, as discussed above. These thiol/disulfide-dependent changes in envelope conformation seem to depend both on autocatalytic processes or cellular thiol oxidoreductases. A relevant redox pathway for the entry of such viruses into cells is the cell surface thiol redox pool of protein disulfide isomerases [reviewed by Tanaka et al., 2020]. The main pool of these abundantly expressed redox chaperones is located at the endoplasmic reticulum, while a small fraction undergoes relocation at the cell surface or is secreted at the extracellular milieu. This so-called pecPDI (peri/epicellular PDI) pool corresponds to ca. 2% of the total pool in endothelial cells [Araujo et al., 2017], however it has been increasingly shown to play important functions in cell adhesion, metalloproteinase regulation, platelet activation, thrombosis and vascular remodeling, among other effects [rev. by Tanaka et al., 2020]. Several evidences indicate that a number of viruses requires pecPDI for cell internalization. Typically, PDI-dependent disulfide reduction of the gp120 viral protein [Fenouillet et al., 2007] or of beta1/beta3 integrins [Wan et al., 2012] mediate viral entry of HIV or dengue viruses, respectively. On the other hand, as commented above, a similar pathway has not been shown for influenza viruses, which do not seem to require pecPDI (nor redox processes at all) for cell internalization. Even so, probably due to additional pathways during viral infection, PDIs have been proposed as therapeutic targets for influenza A and B viruses on the basis of significant prevention of viral replication by a number of compounds known (although nonspecifically) to inhibit PDI, such as juniferdin, 16F16, PACMA31, isoquercetin, epigallocatechin-3-gallate or nitazoxanide. Also, PDI silencing by siRNAs significantly inhibited viral replication [Kim & Chang, 2018]. It is important to note that the concentration of these compounds may be higher than the usual levels that can be attained in vivo and, in addition, SARS Cov-2 was not tested in these studies.
Viral cysteine proteases as potential drug targets
Some other viral proteins with a redox-dependent component have been investigated as putative targets of antiviral drugs. The two cysteine proteases, protease M (Mpro), also referred as 3C-like protease (3CLpro), and the papain-like protease (PLpro) are the main redox dependent anti- SARS-CoV-2 targets studied so far. In these proteases, the catalytic Cys145 (Mpro) or Cys112 (PLpro) exert a nucleophilic attack to the carbonyl group of the scissile peptide bond. The Mpro performs an extensive proteolytic processing of the viral overlapping polyproteins, pp1a and pp1ab, required for viral replication and transcription [Zhou et al., 2020]. The PLpro hydrolyses the non-structural protein (nsp) sequence to the shorter nsp1, nsp2, nsp3 and nsp4 proteins [Han et al., 2005], also essential for viral replication. PLpro can also deubiquitinate or deISGylate host cell proteins, resulting in immune suppression.
Because of its important role in viral cycle and given the absence of closely related homologues in humans, Mpro is an attractive target for antiviral drugs [Pillaiyar et al., 2016]. Alkylation of Mpro Cys145 by Michael acceptors potently inhibits enzyme activity and viral replication in mammal cells [Jin et al., 2020]. Noteworthy, despite the absence of Mpro homologues in humans, covalent bond by Michael acceptors can be quite unspecific and there is a high chance that these compounds affect a broad range of mammal proteins. Therefore, assays that prove target specificity and rule out side effects are imposed. Two relevant limitations for these SARS-CoV-2 assays are the need for a NB3 security level laboratory and the lack of infectivity in wild-type mice, restraining animal models for in vivo studies.
To minimize unspecific and side-effects of thiol-alkylating compounds, some studies have combined virtual screening, structural analyses and functional assays to select compounds that fit and bind with high affinity to the catalytic cleft of these proteases. By using this combination, it was shown that the GC-376, a pre-clinical drug against feline infectious peritonitis [Kim et al., 2016], extensively networks hydrogen bonds with an excellent geometric complementarity to the Mpro active site, making the covalent bond between the GC-376 aldehyde bisulfite and Cys145 [Ma et al., 2020] thermodynamically favorable. The less selective compound disulfiram, clinically used as an anti-alcoholism drug, inhibits both Mpro and PLpro by forming a mixed disulfide between the molecule and the catalytic cysteines [Lin et al., 2018; Xu et al., 2020]. This mechanism can be less efficient since the disulfide bond could be undone by host reducing agents. Disulfiram also forms disulfide bonds with the non-catalytic Cys128 in Mpro and Cys271 in PLpro but with slower kinetics [Lin et al., 2018; Xu et al., 2020]. The compound has an additional mechanism of inhibition by the displacement of the Zn2+ from zinc fingers, altering protein stability [Lin et al., 2018]. α,β-unsaturated esters were also demonstrated to inhibit SARS-CoV-2 PLpro. The nucleophilic attack of the catalytic cysteine forms a covalent thioether with the β carbon [Rut et al., 2020]. Of note, Mpro has eleven other cysteines beyond the catalytic Cys145, but their role in protein structure and function are much less studied, leaving plenty of room for investigations in this field.
Host factor immune response: a plethora of redox pathways
In addition to virus-specific pathways involved in infection, a large number of redox processes — of which we provide only a rough overview —regulate the host immune response at essentially every level.
First, viruses can subvert the host redox environment to their advantage during cell invasion and intracellular replication. A remarkable example is the widely prevalent group of large nucleocytoplasmic DNA viruses (including Poxviruses, Iridoviruses, Mimiviruses and several others). These viruses display Erv-type sulfhydril oxidases, in addition to thioredoxins and in some cases glutaredoxin and other dithiol CysXX(X)Cys enzymes, which together can induce oxidative protein folding in the host cell cytosol [Hakim & Fass, 2010]. In line with the proposed ancestral evolutionary role of these viruses, it is possible that their induced redox protein folding may have predated the oxidative protein folding in the eukaryotic endoplasmic reticulum [rev. by Hakim & Fass, 2010]. Whether similar processes occur for other viruses is unknown.
Some noteful redox-dependent events can regulate immune response to virus infection. Extracellular secretion of low molecular weight thiols such as glutathione (GSH) governs effector T cell responses through decreases in surface redox potentials, the so-called "reductive remodeling strategy" of immune regulation [Yan & Banerjee, 2010]. Similarly, secreted Trx [Plugis et al., 2018] or PDIA1 [Curbo et al., 2009] promote, via disulfide reduction, inactivation or impaired receptor binding of the regulatory cytokine IL-4. In general, cell-surface or extracelllular thiol oxidoreductases can reduce specific thiol targets to activate immune responses [rev. by Tanaka et al., 2020]. In addition, redox-sensitive lysosomal cathepsins are proteases required for SARS-CoV-2 entry. The broad cathepsin inhibitor E64D (inhibits cathepsin B, H, L, and calpain) completely blocked SARS-CoV-2 infection into HEK293 expressing ACE2. A 70% decrease in infection was also achieved by isolated inhibition of cathepsin L, but not of cathepsin B [Ou et al., 2020]. Another interesting protein is GILT (gamma-interferon-inducible lysosomal thiol reductase), which (as its name says) is a dithiol Cys-X-X-Cys-containing protein induced in lysosomes by gamma-interferon during viral infection. GILT was shown to restrict the infection by distinct viruses including SARS-CoV. Interestingly, the restrictive effect was dependent on its lysosomal localization triggered by N-glycosylation, which was abrogated by loss in GILT thiol reductase motifs [Chen et al., 2019]. Moreover, in line with roles of cell-surface protein disulfide isomerases in cell entry, PDIA3 from lung epithelial cells exerts a key role in influenza-A infection by assisting the correct redox folding of viral hemaglutinin during its passage through the host endoplasmic reticulum. Inhibition or silencing of PDIA3 significantly diminishes viral burden and lung immunoinflammatory responses in vivo [Chamberlain et al., 2019].
Last but not least, it is important to point that the above highlights are only a few remarks amid a much more complex redox signaling network spanning the entire immunoinflammatory landscape. This includes many redox-modulated components that will not be covered here such as immune receptors, intracellular post-translational modifications, proteolytic events, transcription factors, gene transcription, extracellular matrix remodeling, cell adhesion, and several others. Likewise, we will not deepen our discussion about Nox NADPH oxidases, only to mention the known important roles of Duoxes in lung epithelial cell defense against influenza A viruses [Vlahos & Selemidis, 2014]. Nox2 has been involved in the formation of neutrophil extracellular traps (NETs), which seem particularly important in the response to SARS-Cov-2 and in the transition from a homeostatic pattern of infection to an uncontrolled inflammatory response [Veras et al., 2020]. Finally, increasing evidence implicate mitochondria in the control of inflammatory cell activation, via redox processes, small intermediates or metabolic reprogramming [Pålsson-McDermott & O’Neill, 2020].
Conclusion and therapeutic perspectives
As briefly discussed here, redox processes involved in viral and more specifically in SARS-CoV-2 infection are complex, multilevel and comprise a number of interconnected oxidizing as well as reducing events. Therefore, it is unlikely that one redox-active compound or intervention will provide a "magic bullet" to mitigate Covid-19. Rather, a mechanism-based target-directed strategy may exploit some "redox Achilles heels" of SARS-CoV-2 at several levels or improve the host inflammatory response. Despite the massive worldwide rush towards drug repurposing, which included a number of redox-active compounds, a good candidate has yet to emerge. Novel redox interventions, therefore, should rely on designing new compounds, peptides, nanobodies or antibodies directed to specific proteins or pathways. Modulation of Nox NADPH oxidases or mitochondrial-associated responses may also contribute to mitigate an inappropriate inflammatory response. Despite these challenges, strategies using small compounds, when used under a rational systems-based approach, might provide interesting perspectives in immune response modulation. A remarkable example is the recent identification in T-cells of >3,000 cysteine residues able to covalently bind natural or newly-designed small electrophiles. Such cysteine targets covered structurally diverse proteins, some with crucial functions in immune response, leading to the identification of several electrophilic compounds able to modulate T-cell activation through distinct mechanisms, highlighting their potential as chemical probes or therapeutic agents [Vinogradova et al., 2020].
As always, successful therapeutic strategies must emanate from basic science studies to reveal in-depth aspects of SARS-CoV-2-related redox pathobiology and cellular/systemic immunoinflammatory responses. Even with the need to urgently fight Covid-19, rigorous preclinical studies on proposed agents cannot be shortcut. The general rule is that the time invested in these studies will pay itself on the long-run in the form of an overall faster track towards secure and effective interventions, even for agents generally regarded as safe.
Francisco R. M. Laurindo¹ and Flávia C. Meotti², Editors
¹Heart Institute (InCor), University of São Paulo Medical School, Brazil
²Institute of Chemistry, University of São Paulo, Brazil
References
- Araujo, T. L.; Zeidler, J. D.; Oliveira, P. V.; Dias, M. H.; Armelin, H. A.; Laurindo, F. R. Protein disulfide isomerase externalization in endothelial cells follows classical and unconventional routes Free Radical Biology and Medicine, 103: 199–208, 2017. » doi: 10.1016/j.freeradbiomed.2016.12.021
- Chamberlain, N.; Korwin-Mihavics, B. R.; Nakada, E. M.; Bruno, S. R.; Heppner, D. E.; Chapman, D. G.; Hoffman, S. M.; Vliet, A. van der; Suratt, B. T.; Dienz, O.; et al. Lung epithelial protein disulfide isomerase A3 (PDIA3) plays an important role in influenza infection, inflammation, and airway mechanics Redox Biology, 22: 101129, 2019. » doi: 10.1016/j.redox.2019.101129
- Chen, D.; Hou, Z.; Jiang, D.; Zheng, M.; Li, G.; Zhang, Y.; Li, R.; Lin, H.; Chang, J.; Zeng, H.; et al. GILT restricts the cellular entry mediated by the envelope glycoproteins of SARS-CoV, Ebola virus and Lassa fever virus Emerging Microbes & Infections, 8(1): 1511–23, 2019. » doi: 10.1080/22221751.2019.1677446
- Curbo, S.; Gaudin, R.; Carlsten, M.; Malmberg, K.; Troye-Blomberg, M.; Ahlborg, N.; Karlsson, A.; Johansson, M.; Lundberg, M. Regulation of interleukin-4 signaling by extracellular reduction of intramolecular disulfides Biochemical and Biophysical Research Communications, 390(4): 1272–7, 2009. » doi: 10.1016/j.bbrc.2009.10.134
- Fenouillet, E.; Barbouche, R.; Jones, I. M. Cell Entry by Enveloped Viruses: Redox Considerations for HIV and SARS-Coronavirus Antioxidants & Redox Signaling, 9(8): 1009–34, 2007. » doi: 10.1089/ars.2007.1639
- Hakim, M. & Fass, D. Cytosolic Disulfide Bond Formation in Cells Infected with Large Nucleocytoplasmic DNA Viruses Antioxidants & Redox Signaling, 13(8): 1261–71, 2010. » doi: 10.1089/ars.2010.3128
- Han, Y.; Chang, G.; Juo, C.; Lee, H.; Yeh, S.; Hsu, J. T.; Chen, X. Papain-Like Protease 2 (PLP2) from Severe Acute Respiratory Syndrome Coronavirus (SARS-CoV): Expression, Purification, Characterization, and Inhibition Biochemistry, 44(30): 10349–59, 2005. » doi: 10.1021/bi0504761
- Hati, S. & Bhattacharyya, S. Impact of Thiol–Disulfide Balance on the Binding of Covid-19 Spike Protein with Angiotensin-Converting Enzyme 2 Receptor ACS Omega, 5(26): 16292–8, 2020. » doi: 10.1021/acsomega.0c02125
- Jain, S.; McGinnes, L. W.; Morrison, T. G. Role of Thiol/Disulfide Exchange in Newcastle Disease Virus Entry Journal of Virology, 83(1): 241–9, 2008. » doi: 10.1128/jvi.01407-08
- Jin, Z.; Du, X.; Xu, Y.; Deng, Y.; Liu, M.; Zhao, Y.; Zhang, B.; Li, X.; Zhang, L.; Peng, C.; et al. Structure of Mpro from SARS-CoV-2 and discovery of its inhibitors Nature, 582(7811): 289–93, 2020. » doi: 10.1038/s41586-020-2223-y
- Kim, Y. & Chang, K. Protein disulfide isomerases as potential therapeutic targets for influenza A and B viruses Virus Research, 247: 26–33, 2018. » doi: 10.1016/j.virusres.2018.01.010
- Kim, Y.; Liu, H.; Kankanamalage, A. C. Galasiti; Weerasekara, S.; Hua, D. H.; Groutas, W. C.; Chang, K.; Pedersen, N. C. Reversal of the Progression of Fatal Coronavirus Infection in Cats by a Broad-Spectrum Coronavirus Protease Inhibitor PLOS Pathogens, 12(3): e1005531, 2016. » doi: 10.1371/journal.ppat.1005531
- Lavillette, D.; Barbouche, R.; Yao, Y.; Boson, B.; Cosset, F.; Jones, I. M.; Fenouillet, E. Significant Redox Insensitivity of the Functions of the SARS-CoV Spike Glycoprotein Journal of Biological Chemistry, 281(14): 9200–4, 2006. » doi: 10.1074/jbc.m512529200
- Lin, M.; Moses, D. C.; Hsieh, C.; Cheng, S.; Chen, Y.; Sun, C.; Chou, C. Disulfiram can inhibit MERS and SARS coronavirus papain-like proteases via different modes Antiviral Research, 150: 155–63, 2018. » doi: 10.1016/j.antiviral.2017.12.015
- Ma, C.; Sacco, M. D.; Hurst, B.; Townsend, J. A.; Hu, Y.; Szeto, T.; Zhang, X.; Tarbet, B.; Marty, M. T.; Chen, Y.; et al. Boceprevir, GC-376, and calpain inhibitors II, XII inhibit SARS-CoV-2 viral replication by targeting the viral main protease Cell Research, 30(8): 678–92, 2020. » doi: 10.1038/s41422-020-0356-z
- Ou, X.; Liu, Y.; Lei, X.; Li, P.; Mi, D.; Ren, L.; Guo, L.; Guo, R.; Chen, T.; Hu, J.; et al. Characterization of spike glycoprotein of SARS-CoV-2 on virus entry and its immune cross-reactivity with SARS-CoV Nature Communications, 11: 1620, 2020. » doi: 10.1038/s41467-020-15562-9
- Pålsson-McDermott, E. M. & O’Neill, L. A. J. Targeting immunometabolism as an anti-inflammatory strategy Cell Research, 30(4): 300–14, 2020. » doi: 10.1038/s41422-020-0291-z
- Petit, C. M.; Chouljenko, V. N.; Iyer, A.; Colgrove, R.; Farzan, M.; Knipe, D. M.; Kousoulas, K. Palmitoylation of the cysteine-rich endodomain of the SARS–coronavirus spike glycoprotein is important for spike-mediated cell fusion Virology, 360(2): 264–74, 2007. » doi: 10.1016/j.virol.2006.10.034
- Pillaiyar, T.; Manickam, M.; Namasivayam, V.; Hayashi, Y.; Jung, S. An Overview of Severe Acute Respiratory Syndrome–Coronavirus (SARS-CoV) 3CL Protease Inhibitors: Peptidomimetics and Small Molecule Chemotherapy Journal of Medicinal Chemistry, 59(14): 6595–628, 2016. » doi: 10.1021/acs.jmedchem.5b01461
- Plugis, N. M.; Weng, N.; Zhao, Q.; Palanski, B. A.; Maecker, H. T.; Habtezion, A.; Khosla, C. Interleukin 4 is inactivated via selective disulfide-bond reduction by extracellular thioredoxin Proceedings of the National Academy of Sciences, 115(35): 8781–6, 2018. » doi: 10.1073/pnas.1805288115
- Ponnusamy, R.; Moll, R.; Weimar, T.; Mesters, J. R.; Hilgenfeld, R. Variable Oligomerization Modes in Coronavirus Non-structural Protein 9 Journal of Molecular Biology, 383(5): 1081–96, 2008. » doi: 10.1016/j.jmb.2008.07.071
- Rut, W.; Lv, Z.; Zmudzinski, M.; Patchett, S.; Nayak, D.; Snipas, S. J.; Oualid, F. El; Huang, T. T.; Bekes, M.; Drag, M.; et al. Activity profiling and structures of inhibitor-bound SARS-CoV-2-PLpro protease provides a framework for anti-COVID-19 drug design bioRxiv, 2020.04.29.068890, 2020. Preprint. » doi: 10.1101/2020.04.29.068890
- Tanaka, L. Y.; Oliveira, P. V.; Laurindo, F. R. Peri/Epicellular Thiol Oxidoreductases as Mediators of Extracellular Redox Signaling Antioxidants & Redox Signaling, 33(4): 280–307, 2020. » doi: 10.1089/ars.2019.8012
- Veras, F. P.; Pontelli, M.; Silva, C.; Toller-Kawahisa, J.; Lima, M. de; Nascimento, D.; Schneider, A.; Caetite, D.; Rosales, R.; Colon, D.; et al. SARS-CoV-2 triggered neutrophil extracellular traps (NETs) mediate COVID-19 pathology MedRxiv, 2020.06.08.20125823, 2020. Preprint. » doi: 10.1101/2020.06.08.20125823
- Vinogradova, E. V.; Zhang, X.; Remillard, D.; Lazar, D. C.; Suciu, R. M.; Wang, Y.; Bianco, G.; Yamashita, Y.; Crowley, V. M.; Schafroth, M. A.; et al. An Activity-Guided Map of Electrophile-Cysteine Interactions in Primary Human T Cells Cell, 182(4): 1009–26.e29, 2020. » doi: 10.1016/j.cell.2020.07.001
- Vlahos, R. & Selemidis, S. NADPH Oxidases as Novel Pharmacologic Targets against Influenza A Virus Infection Molecular Pharmacology, 86(6): 747–59, 2014. » doi: 10.1124/mol.114.095216
- Wan, S.; Lin, C.; Lu, Y.; Lei, H.; Anderson, R.; Lin, Y. Endothelial cell surface expression of protein disulfide isomerase activates β1 and β3 integrins and facilitates dengue virus infection Journal of Cellular Biochemistry, 113: 1681-91, 2012. » doi: 10.1002/jcb.24037
- Wong, S. K.; Li, W.; Moore, M. J.; Choe, H.; Farzan, M. A 193-Amino Acid Fragment of the SARS Coronavirus S Protein Efficiently Binds Angiotensin-converting Enzyme 2 Journal of Biological Chemistry, 279(5): 3197–201, 2004. » doi: 10.1074/jbc.c300520200
- Xiao, X.; Chakraborti, S.; Dimitrov, A. S.; Gramatikoff, K.; Dimitrov, D. S. The SARS-CoV S glycoprotein: expression and functional characterization Biochemical and Biophysical Research Communications, 312(4): 1159–64, 2003. » doi: 10.1016/j.bbrc.2003.11.054
- Xu, L.; Tong, J.; Wu, Y.; Zhao, S.; Lin, B. Targeted Oxidation Strategy (TOS) for Potential Inhibition of Coronaviruses by Disulfiram — a 70-Year Old Anti-Alcoholism Drug ChemRxiv,11936292.v1: 2020. Preprint. » doi: 10.26434/chemrxiv.11936292.v1
- Yan, Z. & Banerjee, R. Redox Remodeling as an Immunoregulatory Strategy Biochemistry, 49(6): 1059–66, 2010. » doi: 10.1021/bi902022n
- Zhou, P.; Yang, X.; Wang, X.; Hu, B.; Zhang, L.; Zhang, W.; Si, H.; Zhu, Y.; Li, B.; Huang, C.; et al. A pneumonia outbreak associated with a new coronavirus of probable bat origin Nature, 579(7798): 270–3, 2020. » doi: 10.1038/s41586-020-2012-7
Add new comment